Jens-Christian Meiners studies tissue damage in spinal cord DCS.
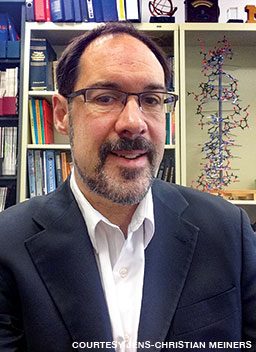
Jens-Christian Meiners, Ph.D., a professor of physics and biophysics at the University of Michigan, focuses his laboratory’s research on mechanics of biological systems, primarily the dynamical properties of DNA and DNA–protein complexes. Meiners and his team are currently working on tissue mechanics and tissue damage through gas bubbles in the spinal cord, or spinal cord decompression sickness (DCS), which won him the 2019 DAN/R.W. “Bill” Hamilton Memorial Dive Medicine Research Grant.
Originally from Hanover, Germany, Meiners studied at the University of Konstanz and University of Bayreuth and graduated from the University of Delaware in 1997 with a master’s degree in physics. He returned to Germany to finish his doctoral degree in polymer physics on the micro and nanoscale using optical techniques and then accepted a postdoctoral position at California Institute of Technology (Caltech) in Pasadena, where his work started to encompass biophysics.
How did your career move into the biological side of physics?
Meiners: At Caltech we worked with single DNA molecules. With the technology of 20 years ago we could mechanically manipulate single DNA molecules using focused laser beams to pull them and change their structure. We wanted to understand how the topology of a DNA molecule affected the dynamics of the molecule, its interaction with other molecules like regulatory proteins and ultimately its biological functions. I got good at manipulating DNA and was even able to tie the molecules into knots — a feat that made a bit of a splash.
In 2000 I became an assistant professor of physics and biophysics at the University of Michigan. As my scientific focus continued to evolve, I became interested in the role of mechanics in biological processes. This approach became the overarching theme, initially with DNA and DNA–protein complexes and later with cellular mechanics. I looked at how mechanical tension in the DNA can turn genes on and off, for instance. I have since worked my way up through the ranks, which included seven years as chair of the biophysics department. After my tenure as chair ended, I was in charge of a well-established lab and had a secure position, so I started to look for what fun and interesting things I could do.
How did you get into diving and dive research?
I started diving about eight years ago, initially on a whim, during a surf trip to the Big Island of Hawai’i. We didn’t have any waves, but a dive shop was offering Discover Scuba dives on the reef, and that was it. I fell in love with diving and got certified. Soon I started to read the published literature on DCS and the proposed mechanisms. I didn’t find as much as I expected about the connection between bubbles, symptoms and the disease. What we started with was, “Bubbles form, [black box], you develop symptoms.” While there were some early studies in decompressed gels, they couldn’t make the connection to biological tissues. Then there were studies in decompressed live animals that showed empirically some tissue damage, but most of the findings didn’t answer my initial question about the mechanism behind it. Something in between was missing, so I decided to poke around, involve some undergraduate students in the project, and see if we could find more precise answers to what happens in that black box.
What was your approach to opening the black box?
We started on the molecular level but couldn’t find anything by looking at whether gas molecules could denature proteins during rapid decompression. Some proteins such as myoglobin have large gaps on the inside where we expect to see gas molecules. In X-ray crystallography, xenon gas in the molecules’ pockets are markers used to map the molecules. We thought that if we put in enough nitrogen or helium during compression that we might rupture the molecules during decompression, which could certainly cause a bad outcome. But despite very high pressures (100 atmospheres), nothing happened. It was a finding, but not the one we had anticipated.
The next approach was to look on the cellular scale for the mechanism that was killing the cells. The literature suggested that contact with micro gas bubbles can kill endothelial cells, but those are technically difficult experiments. We started by making gels and decompressing them to make bubbles form spontaneously, replicating early decompression experiments to see if our findings were the same. Our findings were a bit of an awakening: We saw the bubbles formed during decompression were tearing apart the gels. The understanding in the literature we had studied was that bubbles elastically deformed the gel or tissue, like a balloon inflating and deflating. According to this balloon model, things would eventually go back to their previous shape, meaning any tissue deformation would be reversible if you could shrink the bubbles, like in recompression therapy. This was not the case in our experiments.
Our hypothesis now was the permanent damage we observed in the gels would also happen in tissues. It’s comparable to small paper cuts caused by expanding bubbles in the tissue — the bubbles tear the tissue and do not just block blood flow through a reversible deformation.
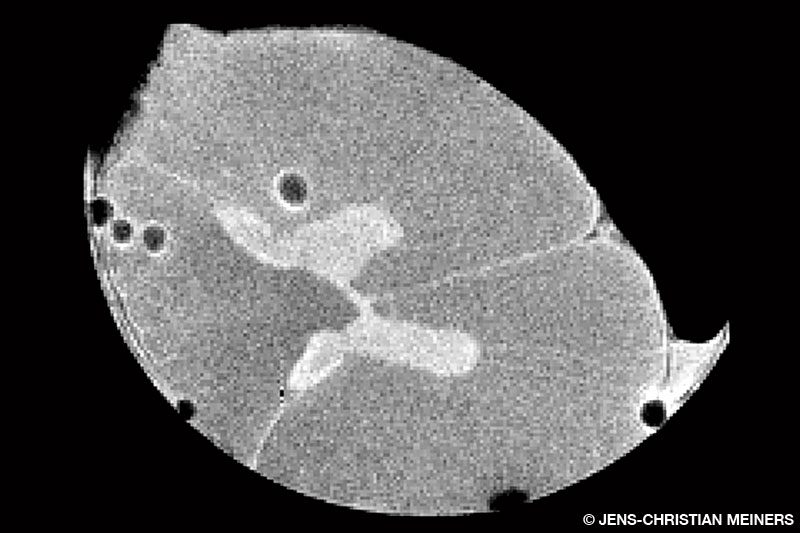
Is this hypothesis the project for which you applied for DAN’s Hamilton research grant?
Yes, we wanted to look at what would happen in actual tissues and chose to work with the spinal cord, mostly due to the severity of reported symptoms and lasting impairment in spinal cord DCS. We used cow spinal cords as a model since they have mechanical properties that are reasonably close to human spinal cords and are readily available from a local butcher. We partnered with one of our MRI imaging experts, Joan Greve (who is also a diver), and built a small, experimental compression chamber that we could place inside the animal MRI scanner.
We got the data that we needed, and our analysis shows good support for our hypothesis that we see irreversible damage to the tissue. Whether it is tearing or a slow viscoelastic deformation, like what you see in Silly Putty, we can’t quite tell. Our experiments ended in an irreversible deformation of the tissue and contradicted the flexible balloon theory.
What is the next step after publishing these findings?
When we performed these experiments we also noticed that bubble formation was rare. We had to go through many samples to find a bubble, even though the profile we used was very aggressive. Saturating the tissue to 7 atmospheres over a day and then rapidly decompressing it led to minimal spontaneous bubble formation, which was surprising and led us to hypothesize that the missing ingredient, active circulation, may be responsible.
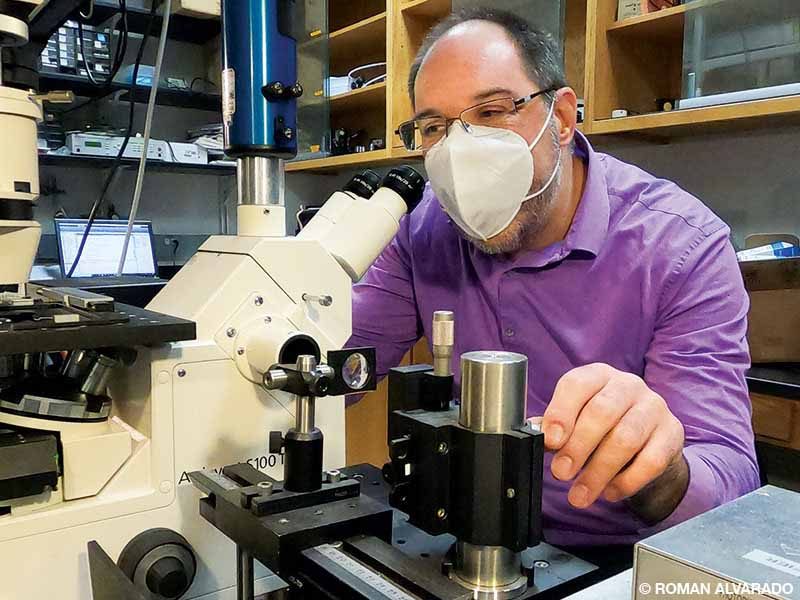
We believe that the key to damaging bubble formation is their nucleation process. Ordinarily, bubbles cannot form spontaneously, at least not easily, unless we are talking about a depth comparable to the Mariana Trench. We know very little about where the gas nuclei originate. Our hypothesis at this point is that nano- and micronuclei are constantly forming in the bloodstream, possibly in areas where a lot of flow and turbulence are present. These nuclei might be stable, and when they transport into the capillaries and the venous system, they experience lower pressure and begin to grow.
This hypothesis needs testing, but it is a reasonable explanation for the absence of large amounts of bubbles in our nonliving tissue samples. Additionally, we might be crushing some of those preexisting nuclei during the compression process in our experiments. The logical next step would be animal experiments to validate this hypothesis in vivo.
Are you working on the next steps?
We are equipped and eager to try it. We have all the facilities, but animal experiments need preparation, such as developing the protocols and applying for ethical approval and additional funding. Our goal is to get to a fundamental understanding of traumatic spinal cord injury mechanics from growing gas bubbles. Our hope is to improve treatment protocols for neurological DCS.

What do you do when you’re not performing physics or dive research?
Diving has become one of my greatest passions. I started diving relatively late, but after that initial Discover Scuba class, I got hooked and progressed quickly into more advanced diving. During a dive holiday in Mexico I tried cave diving — again more by chance than planning — and went through all the training. Those caves have become my favorite dive spots. If I had to pick one, it would probably be Cenote Caterpillar in Muyil, Mexico. I also dive locally in the Great Lakes. We have some beautiful shipwrecks, but I have discovered that I like rocks better than steel.
When I’m not diving, I enjoy all things outdoors, such as trail running or kayaking in the beautiful nature we have in Michigan. I’m also happy just firing up the grill in my backyard.
Explore More
Watch a video featuring Jens-Christian Meiners discussing nanomedicine on “Saturday Morning Physics,” presented by the University of Michigan.
© Alert Diver — Q1 2021